Science News
Delivering science and clinical guidance you can trust
View all Science News AHA members receive important science when it publishes via Science News emails. A weekly edition offers top news from the previous week. Use the subscription page to opt in for the weekly edition and to add topics beyond those associated with your cardiovascular specialty and council.
Recent News
AHA Clinical Update Slides
-
Clinical Update Slide Set: 2025 Guideline for the Management of Patients with Acute Coronary Syndromes (PPTX)
Published: February 27, 2025 -
Clinical Update Slide Set: 2024 Guideline for the Primary Prevention of Stroke (PPTX)
Published: October 21, 2024 -
Clinical Update Slide Set: 2024 Guideline for Perioperative Cardiovascular Management for Noncardiac Surgery (PPTX)
Published: September 24, 2024 -
Clinical Update Slide Set: 2024 AHA/ACC Guideline on the Management of Lower Extremity Peripheral Artery Disease (PPTX)
Published: May 14, 2024 -
Clinical Update Slide Set: 2024 Hypertrophic Cardiomyopathy Guideline (PPTX)
Published: May 8, 2024
Science News Commentaries
Upcoming Meetings
BCVS Scientific Sessions 2025
July 23–26, 2025Marriott Baltimore Waterfront | Baltimore, Maryland
Hypertension Scientific Sessions 2025
September 4–7, 2025Marriott Baltimore Waterfront | Baltimore, Maryland
Scientific Sessions 2025
November 7–10, 2025Ernest N. Morial Convention Center | New Orleans, Louisiana
Resuscitation Science Symposium 2025
November 8–9, 2025New Orleans, Louisiana
View all meetings
Device and Drug Alerts
AHA Journals CME
AHA Journals CME has improved article-CME access for AHA Members. Just 1-Click, from the Online Table of Contents. Learn more.
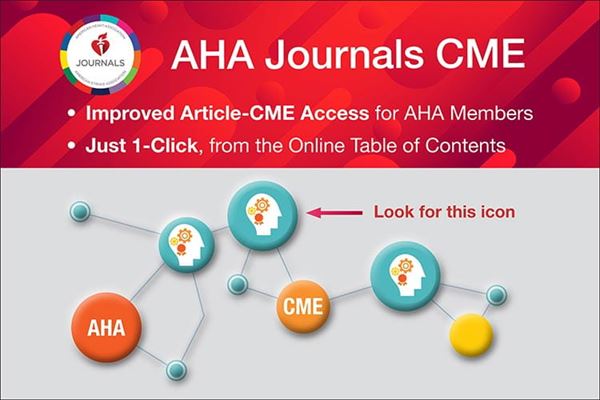